Introduction: Environmental Challenges and Biomechanical Evolution
As Part 2 of the ongoing series Biomechanics of Evolution, this installment delves into the diverse and complex ways organisms have adapted their structures and movements to survive under extreme environmental pressures. Evolution has shaped species across the planet, each biome and habitat offering unique challenges that push the boundaries of biomechanical innovation. From the crushing depths of the deep sea to the arid heat of the desert and the freezing expanse of polar regions, life has evolved unique, highly specialized biomechanical adaptations that, while advantageous, often come with distinct trade-offs.
In Part 1, we discussed how evolution optimizes movement and structure across species, revealing patterns of adaptation through natural selection and biomechanical efficiency. Here, the focus shifts to exploring how environmental pressures drive more intricate adaptations, examining the constant battle between energetic efficiency and physical resilience. Organisms develop solutions that allow them to move efficiently, but often at the expense of other functions. These evolutionary trade-offs manifest in various ways, from the fine motor skills and bipedalism of humans to the sleek, streamlined forms of deep-sea creatures that balance flexibility with pressure resistance.
The environmental pressures of extreme habitats demand biomechanical ingenuity. For instance, the crushing depths of the ocean force aquatic creatures to evolve flexible bodies capable of withstanding immense pressure, while desert animals must navigate the challenges of water conservation and heat regulation. Similarly, creatures inhabiting cold climates develop adaptations to retain heat without sacrificing the mobility needed for survival in barren, frozen landscapes. As diverse as these adaptations are, they all reflect the push-pull dynamic of evolution—trading off one capability to optimize another, often at the cost of long-term health or mechanical efficiency.
Further illustrating these trade-offs are examples of evolutionary “leftovers” or vestigial structures. Though no longer necessary for survival, these remnants from ancestral forms—such as wisdom teeth in humans—provide insight into evolutionary history and highlight the constraints imposed on evolution’s path. These structures, while often functionally redundant, persist and sometimes create biomechanical challenges, underscoring evolution’s tendency to build upon existing frameworks rather than starting anew.
In this chapter, we will also explore how energy efficiency drives biomechanical adaptations across species. Whether it’s the endurance-focused bipedalism of human ancestors or the high-speed sprinting abilities of predators like cheetahs, species face the constant struggle of optimizing energy use for survival. This need for energy conservation influences everything from the migration patterns of birds to the hunting strategies of predators. However, the pursuit of efficiency is rarely without compromise, and these biomechanical innovations often come at the cost of fragility, vulnerability to injury, or other physiological limitations.
By comparing the biomechanical adaptations of various species, this section of the series uncovers not only how different organisms have evolved to meet the unique challenges of their environments, but also how humans fit into the broader spectrum of evolutionary innovation. Whether it’s comparing the endurance running of humans to the speed-focused adaptations of other mammals or examining the role of soft tissues and elasticity in movement, understanding these evolutionary principles sheds light on how evolution crafts both remarkable solutions and inevitable compromises.
Ultimately, Part 2 of Biomechanics of Evolution continues to explore the dynamic relationship between form and function in the animal kingdom, offering a deeper understanding of how life evolves under pressure—literally and metaphorically. From vestigial structures to convergent evolution, this segment emphasizes the delicate balance between environmental demands and biomechanical innovation, revealing the intricate and sometimes precarious ways life has adapted to thrive in its ever-changing habitats.
Environmental Pressures and Biomechanical Innovation
Species around the globe have evolved under the influence of specific environmental factors that force them to develop unique biomechanical solutions. Whether it’s navigating the crushing depths of the ocean, surviving the arid deserts, or thriving in frigid climates, each environment presents a set of biomechanical challenges that shape an organism’s anatomy, movement, and overall functionality. Understanding these environmental pressures gives us insight into how animals have adapted to their habitats, often trading off certain traits to excel in others.
Biomechanics of Deep-Sea Creatures
The deep sea presents one of the most extreme environments on Earth, with high pressure, low temperatures, and complete darkness shaping the biomechanics of the creatures that live there. In the deep ocean, pressure increases by approximately 1 atmosphere for every 10 meters of depth, subjecting animals to immense physical forces. To survive these conditions, many deep-sea creatures have developed adaptations that prevent their bodies from being crushed. For example, deep-sea fish often have reduced skeletal structures, which lowers the risk of their bones being damaged by pressure. Some species have flexible, jelly-like bodies that can withstand the immense pressure without breaking down.
One of the most striking examples of deep-sea adaptation is seen in the giant squid. With no hard skeletal structure, the squid’s body can tolerate the high-pressure environment. Its large eyes, some of the biggest in the animal kingdom, help it detect the faintest light in the pitch-black waters of the deep sea, allowing it to hunt effectively in a habitat with limited visual cues.
Desert-Dwelling Animals
On the other end of the spectrum, desert environments impose challenges related to water conservation, thermoregulation, and mobility on shifting sands. Animals like the camel have evolved physiological adaptations to cope with extreme heat and water scarcity. Camels can go for long periods without drinking water because they can withstand higher levels of dehydration than most other animals. Their kidneys are adapted to conserve water by concentrating urine, and their humps store fat that can be metabolized for energy and water when resources are scarce.
In terms of biomechanics, the camel’s feet are adapted to prevent sinking in the sand. Their wide, flat feet distribute their body weight over a larger surface area, allowing them to move more easily on soft, unstable surfaces. This is a prime example of how environmental pressure—in this case, loose, shifting sand—drives specific biomechanical adaptations that allow an animal to thrive.
Cold-Climate Animals
In cold climates, animals face the challenge of maintaining body heat while remaining agile enough to find food and avoid predators. Polar bears are a well-known example of this biomechanical balance. They have a thick layer of blubber and dense fur that insulates them from the freezing temperatures of the Arctic. However, maintaining warmth also requires them to be efficient hunters, especially in an environment where food is scarce.
Polar bears have large paws that allow them to distribute their weight while walking on ice, much like camels on sand. Their powerful muscles enable them to swim long distances in freezing waters to hunt for seals. This combination of thermal insulation and biomechanical strength illustrates how animals in cold climates must balance the trade-offs between staying warm and staying mobile.
Environmental pressures shape the form and function of animals in a wide variety of ways. From the crushing depths of the ocean to the heat of the desert and the cold of the Arctic, species evolve biomechanical solutions that allow them to survive in environments that would otherwise be inhospitable.
Energetic Trade-offs in Evolution
In the world of biomechanics, energy efficiency is critical for survival. Every movement an organism makes costs energy, and over time, natural selection favors adaptations that optimize energy expenditure. However, energy efficiency often comes at a cost, leading to evolutionary trade-offs. For example, animals that are built for endurance may sacrifice speed, while those optimized for speed may not be able to sustain long-distance movement. This section explores how organisms have evolved to manage the balance between energy use, movement, and survival.
Human Ancestors and Energy Foraging
In early human evolution, energy efficiency was paramount for survival. Human ancestors needed to forage and hunt over vast distances to meet their caloric demands, which drove significant biomechanical adaptations. One of the most transformative changes was the evolution of bipedalism—the ability to walk upright on two legs. This adaptation allowed early hominins to travel longer distances more efficiently than their quadrupedal predecessors, expending less energy while covering more ground in search of food and water.
Bipedalism provided several evolutionary advantages. By freeing the hands, it enabled early humans to carry tools, weapons, and gathered resources, which improved their ability to adapt to varying environments. The upright posture also reduced the body’s exposure to direct sunlight during the heat of the day, which was particularly advantageous in open savannahs. Additionally, bipedalism facilitated endurance running, a critical skill for persistence hunting, where early humans would exhaust prey over long distances.
However, the shift to bipedalism was not without its trade-offs. While this mode of locomotion improved energy efficiency, it introduced significant biomechanical challenges. The human spine, originally adapted for quadrupedal movement, had to bear the full weight of an upright torso. To compensate, the spine evolved an S-shaped curve, which improved balance and shock absorption but also made humans more susceptible to lower back pain and spinal injuries. Modern humans frequently experience conditions such as herniated discs and sciatica, which stem from the biomechanical stresses of walking upright.
Similarly, the shift placed increased strain on the hips, knees, and feet. The human pelvis became shorter and wider to stabilize the upper body during bipedal locomotion, but this adaptation narrowed the birth canal, making childbirth more difficult and risky compared to other primates. Knee and hip joints, under constant pressure from supporting body weight, are prone to degenerative conditions such as arthritis. Additionally, the foot’s arch, crucial for absorbing impact and providing propulsion, is susceptible to issues like plantar fasciitis and flat feet.
Despite these drawbacks, bipedalism was a cornerstone of human evolution, offering energy efficiency and versatility that allowed early humans to thrive in diverse environments. It remains a defining feature of our species, reflecting the complex trade-offs inherent in evolutionary biomechanics.
Birds and Long-Distance Migration
Birds provide another example of energy optimization in evolutionary biomechanics. Many bird species, such as the Arctic tern, engage in long-distance migrations that span thousands of miles. To make these journeys, birds have evolved highly efficient flight mechanisms. The shape of their wings, for example, is optimized for gliding and soaring, reducing the amount of energy they expend during flight. Birds like the albatross can cover long distances with minimal flapping, taking advantage of wind currents to conserve energy.
However, not all birds are optimized for endurance. Birds of prey, such as hawks and falcons, have shorter, more muscular wings that allow them to make rapid, powerful movements. While these birds are highly efficient hunters, they sacrifice energy efficiency in long-distance flight for agility and speed.
Energetic Constraints in Predators
Predators face unique energetic challenges, as they must balance the energy expended during the hunt with the calories gained from their prey. Cheetahs, for example, are the fastest land animals, capable of reaching speeds of up to 70 mph in short bursts. However, this speed comes at a significant energy cost. Cheetahs can only sustain their top speed for a matter of seconds before overheating and exhausting their energy reserves.
To compensate, cheetahs rely on stealth and short, explosive bursts of speed to capture prey, conserving energy whenever possible. This represents a clear trade-off between energy efficiency and the biomechanical demands of high-speed hunting.
The Role of Evolutionary “Leftovers” (Vestigial Structures)
Evolution is not always a process of perfect refinement. As species adapt to new environments, certain structures that were once useful may lose their primary function but remain part of the organism’s anatomy. These vestigial structures offer a fascinating glimpse into the evolutionary history of a species and highlight the constraints imposed by evolutionary pathways.
Wisdom Teeth: A Vestigial Challenge in Modern Humans
In humans, one of the most well-known vestigial structures is wisdom teeth. These third molars, which typically emerge in early adulthood, once served an essential purpose in our evolutionary past. Early human ancestors, such as Australopithecus and other hominins, had larger jaws that could comfortably accommodate these additional molars. In those times, diets consisted primarily of tough, fibrous plant material and uncooked foods, which required significant chewing power to process. Wisdom teeth provided the extra surface area needed to grind down hard and coarse food, making them a valuable asset for survival.
However, with the advent of cooking, food preparation, and dietary changes, the role of wisdom teeth diminished. Cooking made food softer and easier to chew, reducing the reliance on large molars for grinding. Over time, human jaws began to shrink as smaller, more compact jaws became sufficient for processing softer diets. This evolutionary shift rendered wisdom teeth increasingly redundant. While our jaws evolved to be smaller, the genetic blueprint for developing a full set of 32 teeth, including wisdom teeth, persisted.
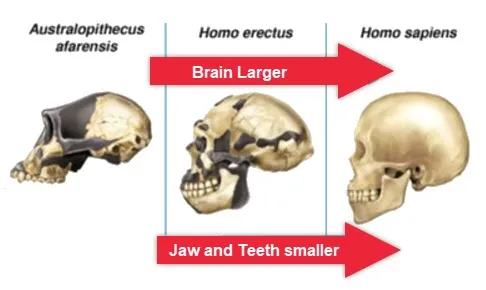
The red arrow labeled “Brain Larger” points to the progressive increase in brain size from Australopithecus afarensis to modern humans. This increase in brain capacity reflects the evolutionary shift toward enhanced cognitive abilities, including problem-solving, communication, and tool use. As hominins evolved, larger brains required more space within the skull, causing significant changes in cranial structure.
The second red arrow, labeled “Jaw and Teeth Smaller,” emphasizes the corresponding reduction in jaw and teeth size. With the braincase expanding, less space remained for the jaw, resulting in smaller teeth and jaws. This adaptation is also linked to dietary changes. As early humans developed tools and began cooking food, the need for large jaws and teeth to process raw, tough materials diminished.
The reduction in jaw size left less room for the third molars, or wisdom teeth, which often become impacted in modern humans. This evolutionary trade-off highlights how human anatomy adapted to prioritize brain development over chewing efficiency.
Overall, this image demonstrates how the interplay between brain growth and jaw reduction reflects a broader trend in human evolution, emphasizing the shift toward enhanced intelligence and cultural adaptation.
In modern humans, the presence of wisdom teeth often creates more problems than benefits. Many people experience issues such as impaction, where the wisdom teeth do not have enough space to erupt fully. Impacted wisdom teeth can lead to a host of complications, including pain, infection, swelling, and damage to adjacent teeth. In severe cases, cysts or tumors can form around the impacted teeth, further complicating oral health. As a result, wisdom tooth extraction has become a common dental procedure, with millions of extractions performed annually worldwide.
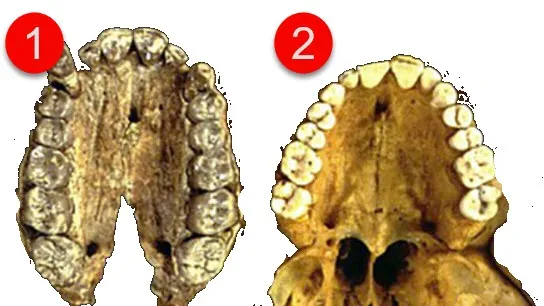
In Australopithecus, the dental arch is broader and more U-shaped, with a longer AP distance reflecting a larger jaw and teeth size. This configuration allowed for the processing of tougher, unprocessed foods, such as raw plants and meat, which required more extensive chewing. The larger jaw also accommodated larger teeth, including molars and canines, essential for a diet reliant on hard-to-digest materials.
In contrast, the modern human palate (2) is narrower and more parabolic, with a shorter AP diameter. This reduction corresponds to smaller jaws and teeth, reflecting significant dietary and evolutionary shifts. As humans developed tools and cooking methods, the mechanical demands on teeth and jaws lessened, leading to smaller dentition and a more refined dental arch. Additionally, the smaller jaw structure in humans is a result of cranial changes that prioritize space for an enlarged braincase.
These differences highlight the evolutionary trade-offs between dietary adaptations and craniofacial morphology. While Australopithecus relied heavily on robust dental features for survival, humans have evolved more efficient cranial structures for cognitive development and cultural advancements, further reducing reliance on large jaws and teeth. This transformation underscores the profound impact of dietary and environmental changes on human evolution.
The persistence of wisdom teeth in modern humans is a clear example of how evolutionary constraints shape anatomy. Evolution is not a process of perfection but rather one of modification. It builds upon existing frameworks rather than starting anew, even when certain features become obsolete. The slow pace of evolutionary change, coupled with the lack of immediate selective pressure to eliminate wisdom teeth entirely, ensures their continued presence in human anatomy.
This phenomenon underscores a broader principle of evolutionary biology: remnants of our ancestral past can linger long after their utility has faded, often creating challenges for modern organisms. Wisdom teeth, while no longer essential, serve as a reminder of humanity’s evolutionary journey and the compromises inherent in our biological development.
The Appendix: A Vestigial Structure with Lingering Functionality
Another classic example of a vestigial structure in humans is the appendix, a small, finger-like pouch attached to the large intestine. In herbivorous ancestors, the appendix played a significant role in digesting cellulose from plant material. Early human diets consisted primarily of raw vegetation, which was tough and required specialized adaptations for digestion. The appendix functioned as part of a larger cecum, housing bacteria that helped break down cellulose and extract nutrients from fibrous plant matter.
Over evolutionary time, as humans transitioned to more diverse diets that included meat and processed foods, the reliance on the appendix for digestion diminished. The advent of cooking further reduced the need for extensive bacterial fermentation of plant material, as cooked foods are easier to chew and digest. Consequently, the human appendix became smaller and largely lost its original function. Despite its reduced role, the appendix persists in modern anatomy, often becoming a source of health complications rather than utility.
One of the most well-known issues associated with the appendix is appendicitis, a potentially life-threatening condition where the appendix becomes inflamed. Appendicitis can occur when the opening of the appendix becomes blocked, often by a small piece of stool, foreign material, or an infection. This blockage can lead to bacterial overgrowth, causing swelling, pain, and sometimes rupture. A ruptured appendix is a medical emergency, as it can release harmful bacteria into the abdominal cavity, leading to severe infection. As a result, surgical removal of the appendix, known as an appendectomy, is one of the most common emergency procedures performed worldwide.
Despite its reputation as a largely useless remnant of evolution, the appendix may not be entirely redundant. Recent studies suggest that it could still serve a secondary function in the human body, particularly in the immune system. The appendix is thought to act as a “safe house” for beneficial gut bacteria. In cases of gastrointestinal illness, such as severe diarrhea, the appendix may help replenish the gut microbiota, aiding in recovery and maintaining digestive health. This potential role highlights the complexity of evolutionary remnants, which can sometimes acquire new or ancillary functions even after losing their primary purpose.
The appendix underscores the intricate and imperfect nature of evolution. While it no longer serves the essential digestive role it did for our ancestors, it remains a fascinating example of how evolutionary history shapes modern anatomy. This small, seemingly insignificant organ reflects the compromises inherent in evolution, where structures are repurposed or diminished rather than eliminated outright, often leaving behind traces of our past in unexpected ways.
The coccyx
The coccyx, commonly known as the tailbone, is one of the most intriguing vestigial structures in the human body. Located at the base of the vertebral column, this small, triangular bone is a remnant of the tail found in our primate ancestors. While humans no longer possess functional tails, the coccyx provides a fascinating glimpse into evolutionary history and serves as a reminder of the shared ancestry among primates and other mammals.
In early primates, tails played an essential role in balance, mobility, and communication. Arboreal species, for instance, relied on their tails for stability and maneuverability while navigating through trees. As human ancestors gradually adopted bipedal locomotion and transitioned to a ground-dwelling lifestyle, the functional need for a tail diminished. Over time, evolutionary pressures favored the reduction of this appendage, leading to its vestigial state in modern humans.
Though the coccyx no longer serves its original purpose, it is not entirely useless. Anatomically, it provides an attachment site for various muscles, tendons, and ligaments, including those involved in pelvic floor support and certain movements of the lower body. For example, the coccygeal muscles and the anococcygeal ligament anchor to the coccyx, contributing to the support of the pelvic organs and assisting in defecation. Additionally, the gluteus maximus, one of the body’s largest muscles, attaches to the coccyx, aiding in movement and posture. While these functions are secondary compared to its original role as part of a tail, they demonstrate how evolution repurposes structures rather than eliminating them entirely.
Despite these minor roles, the coccyx is often associated with pain and injury rather than functionality. A condition known as coccydynia, or tailbone pain, can occur due to trauma, prolonged sitting on hard surfaces, or repetitive stress. In some cases, the pain becomes chronic, significantly impacting quality of life. The susceptibility of the coccyx to injury reflects its reduced functional importance and highlights the compromises inherent in evolutionary biology.
The persistence of the coccyx underscores a broader principle of evolution: natural selection operates on existing structures, modifying them to meet current needs rather than designing entirely new features. This evolutionary constraint explains why vestigial structures like the coccyx, while no longer essential, remain part of the human anatomy. They are evolutionary “leftovers,” relics of a past that continues to shape the present.
The coccyx is a testament to the complexity and imperfection of evolution. While it no longer serves the purpose it once did, it reminds us of our ancestral past and the gradual changes that have shaped the human body. Its presence, though vestigial, reflects the intricate narrative of evolutionary adaptation and compromise.
Comparative Biomechanics Across Species: Human Adaptations in Focus
When comparing biomechanics across species, humans present an especially interesting case, having evolved unique adaptations, particularly bipedalism and fine motor control, which set them apart from other primates. Examining human biomechanics in comparison to other species sheds light on how evolutionary paths diverge and converge based on environmental pressures and survival needs. This section explores how human adaptations compare to other species, especially in terms of locomotion, tool use, and endurance.
Bipedalism in Humans vs. Other Primates
Humans are one of the few species to have evolved bipedalism, walking on two legs as their primary mode of locomotion. This adaptation provided early hominins with significant survival advantages, including freeing the hands for carrying objects and tools, and enabling long-distance travel. In contrast, most other primates, such as chimpanzees and gorillas, remain primarily quadrupedal and knuckle-walk on all fours. While primates can stand and walk briefly on two legs, they do not have the skeletal or muscular structure needed for efficient bipedalism over long distances.
From a biomechanical perspective, bipedalism in humans requires a distinct set of adaptations. The pelvis in humans is shorter and broader than in other primates, allowing for better support of the upper body and stability while walking upright. The spine in humans also has a distinctive S-shaped curve, which acts as a shock absorber and helps maintain balance. In contrast, the spine of a chimpanzee or gorilla is straighter, which is better suited for supporting a quadrupedal stance but not for upright walking.
However, bipedalism comes with trade-offs. The vertical orientation of the human spine increases the risk of lower back pain and disc herniation, as the weight of the upper body puts pressure on the lower vertebrae. Similarly, the shape of the human pelvis, while useful for walking, narrows the birth canal, contributing to the obstetric dilemma, where childbirth is more difficult and risky for humans compared to other primates. These examples illustrate how bipedalism represents a biomechanical trade-off between mobility and structural stability.
Fine Motor Skills in Humans vs. Other Species
Another biomechanical feature that sets humans apart is the development of fine motor skills, particularly the ability to manipulate objects and use tools with precision. While many animals, especially primates, have dexterous hands and can use simple tools, humans possess a level of manual dexterity unmatched in the animal kingdom.
The evolution of the opposable thumb allowed humans to grasp and manipulate objects with precision. This thumb, along with the ability to move the fingers independently, gives humans a unique advantage when it comes to making and using tools. Early humans used this ability to create more complex tools, which likely played a crucial role in survival, allowing them to hunt more efficiently, prepare food, and build shelters.
In contrast, while chimpanzees and other primates also use tools, their hand structure is more adapted for climbing. Their fingers are longer, and their thumb is less opposable, making them more efficient in grasping branches but less adept at precision tasks. This difference illustrates the trade-off between manual dexterity and arboreal agility in evolutionary biomechanics.
The fine motor skills of humans are also reflected in the structure of the shoulder joint, which evolved to allow a wide range of motion for tasks such as throwing. While this mobility is a significant advantage, it also makes the shoulder one of the most injury-prone joints in the human body. Shoulder dislocations and rotator cuff injuries are common in humans, reflecting the biomechanical compromise between mobility and stability in the upper limbs.
Endurance Running in Humans vs. Other Mammals
Humans also possess a unique biomechanical advantage in the form of endurance running. While most animals are faster sprinters than humans, few can match the human ability to run long distances at a steady pace. This trait is thought to have evolved in early humans as part of a strategy known as persistence hunting, where early humans would chase prey over long distances until the animal collapsed from exhaustion.
In comparison to other mammals, such as cheetahs or gazelles, humans are much slower sprinters. Cheetahs, for example, can reach speeds of up to 70 mph, but they can only maintain this pace for short distances before they overheat and must stop. In contrast, humans are built for endurance. Our long legs, arched feet, and Achilles tendons allow us to run efficiently for extended periods without overheating.
Humans also have a unique cooling system. Unlike most animals, which cool down primarily by panting, humans rely on sweating to regulate body temperature during exertion. This cooling mechanism allows humans to continue running even in hot climates, giving them an advantage in endurance over many animals that must stop to cool down.
However, the trade-off for this endurance capacity is increased wear and tear on the joints. Long-distance running places significant strain on the knees, hips, and lower back, leading to conditions such as osteoarthritis in older individuals. While endurance running was an essential survival skill for early humans, it also introduces the risk of chronic joint problems, illustrating the biomechanical trade-off between endurance and joint health.
Constraints of Gravity: How Size and Mass Limit Human Movement
In human biomechanics, gravity plays a crucial role in shaping our movement patterns, posture, and energy expenditure. As a bipedal species, humans constantly contend with the forces of gravity, which impact everything from standing to walking, running, and lifting. Larger body mass in humans leads to greater gravitational forces acting on the body, which in turn influences joint load, muscular effort, and movement efficiency.
Human Examples:
In larger or heavier humans, gravity increases the load on the spine, knees, and hips, making it harder to maintain optimal posture and move efficiently. For example, obesity significantly increases the strain on these joints, often leading to conditions like osteoarthritis. The added weight requires the muscles and bones to work harder to support the body, resulting in wear and tear, reduced agility, and often chronic pain.
On the other hand, smaller and lighter individuals experience less strain from gravity on their skeletal systems, allowing for greater agility and speed. Athletes in sports like gymnastics or rock climbing often have smaller body frames, enabling them to maneuver more easily against gravity with higher strength-to-weight ratios.
Even at a biomechanical level, gravity dictates human locomotion. The Achilles tendon in humans stores elastic energy during walking and running, helping reduce the energy cost of movement. However, as people age or gain weight, this system becomes less efficient due to the increased load, leading to injury risks and a higher energy expenditure to maintain basic movements.
Moreover, pregnancy illustrates a temporary but significant biomechanical challenge with gravity. The additional weight of the fetus shifts a woman’s center of gravity forward, causing changes in posture, such as an exaggerated lumbar curve (lordosis) to compensate. This adaptation can lead to lower back pain due to increased stress on the spine.
Thus, gravity consistently influences how humans move and the constraints imposed on our skeletal and muscular systems. Heavier individuals face more biomechanical challenges in movement and posture compared to lighter ones, and all humans must constantly adapt to this force through muscle and skeletal compensations.
Biomechanical Innovation Through Convergent Evolution
Convergent evolution refers to how different species develop similar traits independently to solve comparable biomechanical challenges. In humans, convergent evolution has played a crucial role in shaping our anatomy and biomechanics in response to environmental pressures and similar needs for survival, such as the evolution of bipedalism, tool use, and efficient thermoregulation.
Human Examples:
One of the most notable examples of convergent evolution in humans and other species is bipedalism. While most mammals move on four limbs, humans evolved to walk on two legs, just like certain birds (e.g., ostriches) and kangaroos. This form of locomotion evolved independently but serves a similar purpose: freeing the upper limbs for tasks like carrying objects and improving energy efficiency during travel over long distances. The trade-off for humans, however, is lower back pain and spinal issues, as our upright posture places more stress on the vertebral column compared to quadrupedal species.
Another example of biomechanical convergence is in the hands. While humans evolved highly dexterous hands capable of precision and grip, similar adaptations can be seen in the octopus and raccoons, both of which have evolved fine motor skills independently. For humans, the development of the opposable thumb and intricate hand muscles allowed for complex tool-making and manipulation, giving early humans a survival advantage. The drawback, however, is vulnerability to repetitive strain injuries like carpal tunnel syndrome, which arise from modern overuse of hands in tasks like typing and other repetitive manual work.
Lastly, thermoregulation is another area where convergent evolution comes into play. Humans sweat to cool down during long-distance running, much like certain desert-dwelling animals like camels. This allows us to persist in hot environments, but the downside is the need for continuous water intake to prevent dehydration, a vulnerability not as prominent in species that rely on different cooling mechanisms, like panting in dogs.
These examples demonstrate how convergent evolution has shaped human biomechanics in response to environmental pressures, leading to both beneficial adaptations and trade-offs.
The Role of Soft Tissue in Biomechanics: Elasticity and Flexibility
Soft tissues—muscles, tendons, and ligaments—play a central role in human biomechanics by providing elasticity and flexibility, allowing for efficient movement. These tissues store energy, facilitate mobility, and enable a wide range of motion, but they also introduce vulnerabilities, such as the risk of injury from overuse or strain.
Human Examples:
In humans, the Achilles tendon is a key example of how soft tissues contribute to energy storage and movement efficiency. During walking and running, the Achilles tendon stretches and recoils, storing and releasing energy with each step. This process reduces the metabolic cost of locomotion, making humans efficient long-distance runners. However, the Achilles tendon is also prone to injury, especially in athletes. Conditions like Achilles tendinitis or rupture are common overuse injuries that occur when the tendon is subjected to repetitive stress without adequate recovery.
Another example of the importance of soft tissues is in the hamstrings and quadriceps, which work together to allow for knee flexion and extension. These muscles and their associated tendons are crucial for activities like sprinting, jumping, and squatting. However, muscle imbalances or improper stretching can lead to hamstring strains or tears, which are some of the most common sports injuries.
Soft tissues also play a role in maintaining joint flexibility. Ligaments, which connect bones, stabilize joints like the knees, shoulders, and ankles. While flexibility is essential for a wide range of motion, excessive flexibility without the corresponding strength can lead to joint instability and an increased risk of dislocations and sprains. This is particularly relevant in hypermobile individuals who are prone to joint-related issues.
Overall, while soft tissues provide humans with the elasticity and flexibility necessary for efficient movement, they are also vulnerable to injury, illustrating the trade-off between flexibility and biomechanical stability.
Evolutionary Remnants: Vestigial Structures and Their Biomechanical Implications
In humans, vestigial structures offer fascinating insights into our evolutionary past, but they also introduce biomechanical challenges and vulnerabilities that have persisted over time.
Human Examples:
One of the most well-known vestigial structures in humans is the appendix, a small pouch attached to the large intestine. In herbivorous ancestors, the appendix played a role in digesting tough plant material, particularly cellulose. However, as human diets evolved to include more varied and processed foods, the appendix lost its digestive function. Today, it serves no significant purpose in most individuals, yet it can become inflamed, leading to appendicitis, a condition that requires surgical removal. The appendix is a clear example of how evolutionary remnants can pose health risks, despite no longer serving their original function.
Another vestigial feature in humans is wisdom teeth. Early human ancestors had larger jaws to accommodate more teeth, which were useful for chewing coarse, fibrous plant material. However, as humans developed tools and cooking methods, the need for extra molars diminished, and jaw sizes shrank. Many modern humans no longer have enough space for wisdom teeth, leading to impaction, infection, and the need for extraction. This evolutionary remnant represents a biomechanical trade-off, as the modern human jaw has evolved for speech and finer control, but at the expense of space for extra molars.
The tailbone, or coccyx, is another vestigial structure that once supported the tails of our primate ancestors. While humans no longer have functional tails, the coccyx remains at the base of the spine. In modern humans, the coccyx has limited function, serving as an attachment site for certain muscles and ligaments involved in sitting. However, trauma to the coccyx can lead to coccydynia, a painful condition that highlights the vulnerability of this evolutionary leftover.
These vestigial structures remind us that evolution is a process of modification rather than perfection. While they no longer serve their original purpose, they persist in modern humans and can sometimes contribute to biomechanical challenges and health issues.
Conclusion
The journey through the Biomechanics of Evolution series, across both parts, has illuminated the extraordinary ways organisms have adapted to survive the relentless pressures of nature. From deep-sea creatures braving crushing depths to humans reshaping their anatomy for endurance and dexterity, evolution is revealed as a master craftsman, balancing the competing demands of form and function. Yet, as much as these adaptations are remarkable, they also underscore the inherent trade-offs and constraints that define life’s progression.
Patterns of Adaptation and Environmental Pressures
In Part 1, we explored how evolution optimizes movement and structure across species, focusing on the principles of natural selection and biomechanical efficiency. These foundational concepts laid the groundwork for understanding how life has evolved to maximize survival while minimizing energy expenditure. From the streamlined forms of aquatic animals to the energy-saving adaptations of human bipedalism, organisms have continuously refined their physical structures to align with environmental demands.
Part 2 extended this exploration to examine how extreme environments, such as the deep ocean, deserts, and polar regions, shape biomechanical innovation. For instance, deep-sea organisms like the giant squid evolved flexible, pressure-resistant bodies to survive where light and stability are nonexistent. Conversely, desert dwellers such as camels developed unique solutions to conserve water and traverse shifting sands. These examples reflect the incredible adaptability of life, yet they also highlight a universal truth: every evolutionary solution comes with a cost.
The Trade-Offs of Evolutionary Ingenuity
A recurring theme in both parts of this series is the trade-offs inherent in biomechanical adaptations. Evolution rarely delivers perfect solutions; instead, it works within the constraints of existing structures, often resulting in compromises. Human bipedalism, for example, allowed early hominins to travel long distances more efficiently, freeing their hands for tool use and other tasks. Yet, this adaptation came at the expense of increased strain on the spine, hips, and knees, leading to modern issues such as lower back pain and arthritis.
Similarly, energy efficiency, a hallmark of evolutionary success, often limits an organism’s ability to perform other critical functions. Predators like cheetahs are optimized for speed, but their high-speed chases are energy-intensive and unsustainable over long periods. These compromises illustrate the delicate balancing act of evolution—enhancing one capability often diminishes another.
Vestigial Structures: Echoes of the Past
The exploration of vestigial structures provided a fascinating lens through which to view evolution’s imperfections. Structures like wisdom teeth and the appendix in humans serve as reminders of our ancestral past, retaining minor or redundant functions that no longer align with modern needs. Yet, these evolutionary “leftovers” also reveal the constraints imposed by evolutionary pathways, as natural selection builds upon preexisting frameworks rather than starting anew. Even as these structures lose relevance, they occasionally acquire secondary roles, exemplifying the complexity and resourcefulness of evolution.
Convergent Evolution and Biomechanical Parallels
Across both parts, the concept of convergent evolution demonstrated how similar environmental pressures lead to analogous solutions in unrelated species. From the endurance running of humans to the gliding capabilities of birds like albatrosses, nature repeatedly finds parallel paths to navigate common challenges. These instances of biomechanical convergence not only highlight the efficiency of evolutionary processes but also underscore the shared constraints faced by all organisms.
Humans: A Case Study in Biomechanical Innovation
Throughout the series, humans have served as a unique case study in evolutionary biomechanics. Our species embodies the profound interplay between adaptation and compromise. Bipedalism, fine motor skills, and endurance running set humans apart from other species, yet they come with vulnerabilities such as injury-prone joints and energy-intensive childbirth. This duality reflects humanity’s evolutionary path—a triumph of adaptation tempered by the limitations of biology.
A Reflection on Evolution’s Legacy
The Biomechanics of Evolution series ultimately underscores the complexity of life’s evolutionary journey. Adaptations are not merely triumphs of ingenuity; they are also reflections of survival’s cost. From the simplest organism to the most advanced, evolution shapes life under the relentless forces of natural selection and environmental pressures, carving out innovations while leaving behind traces of its imperfect artistry.
By examining the biomechanical strategies of various species, we gain a deeper appreciation for the interconnectedness of all life forms. Evolution is a story of resilience and compromise, a tale of life thriving not in spite of its imperfections but because of them. As science continues to uncover the intricate mechanisms of evolution, we are reminded of our place within this grand narrative—an ever-evolving species shaped by the same forces that govern all life on Earth.
References
- “Why do mammals hop? Understanding the ecology, biomechanics and evolution of bipedal hopping”, Journal of Experimental Biology, 2018., This article explores the ecological and biomechanical factors that have led to the evolution of bipedal hopping in mammals. Link
- “7 Vestigial Features of the Human Body”, Britannica, This piece discusses various vestigial structures in humans, including the appendix, wisdom teeth, and the coccyx, providing insights into their evolutionary backgrounds. Link
- “Vestigial Structures: What Are They, Examples”, Osmosis, 2022. This resource offers an overview of vestigial structures, explaining their significance in evolutionary biology and providing examples from human anatomy. Link
- “Integrating biomechanics in evolutionary studies, with examples from the vertebrate limb”, Journal of Experimental Biology, 2023. This article discusses the importance of integrating biomechanics into evolutionary studies, using the vertebrate limb as a case study. Link
- “Climate Effects on Human Evolution”, Smithsonian National Museum of Natural History, 2024. This resource examines how climate change has influenced human evolution, highlighting the role of environmental pressures in shaping human adaptations. Link
- “Vestigial Organs: 7 Body Parts We Don’t Need”. University Health News, 2018. This article lists and describes vestigial organs in the human body, discussing their evolutionary origins and current relevance. Link
- “Convergence, Adaptation, and Constraint”. Evolution, 2011. This paper explores the concepts of convergent evolution, adaptation, and the constraints that influence evolutionary pathways. Link
- “Vestiges of the natural history of development: historical holdovers in the age of molecular biology”. Evolution: Education and Outreach, 2014. This article discusses vestigial structures from a developmental biology perspective, emphasizing their significance in understanding evolutionary processes. Link
- “Molecular Mechanisms Underlying Vertebrate Adaptive Evolution: A Review”. Genes, 2023. This review delves into the molecular mechanisms that drive adaptive evolution in vertebrates, providing a comprehensive overview of current research.. Link
- “5 Vestigial Body Parts Found in Humans”. Discover Magazine, 2023.. This article highlights five vestigial body parts in humans, discussing their evolutionary significance and the reasons they persist. Link